INTRODUCTION
Xenotransplantation of organs from pigs into humans has been considered as a promising solution for shortage of organs, because of their physiological and anatomical similarities. Recently, a significant progress has been made in that cardiac xenograft from the α-1,3-galactosyltransferase knock-out (GT KO) pigs with human complement regulatory protein and human thrombomodulin molecules to baboons survived for over 900 days (Mohiuddin et al., 2016) and renal xenotransplantation extended over 227 days with GT KO/CD55 (decay accelerating factor; DAF) transgenic pig-to-macaque (Higginbotham et al., 2015). However, except these successful reports, many xenograft cases have been suffered from various immune rejections. To minimize immune rejection phenomenon after TG pig-to-primate xenotransplantation, GT KO pigs with an overexpression of and simultaneous inhibition of human complement activation by the introduction of human complement-regulatory genes, such as CD46 (membrane cofactor protein, MCP), CD55 (Lee et al., 2011), and CD59 (Lambrigts et al. 1998; McGregor et al. 2004).
The previous studies have demonstrated that thrombotic microangiopathy had been occurred in primates following xenotransplantation with GT KO pig solid organs without hyperacute immune rejection causing galactose-α-1,3-galactose antigens (α-Gal) (Houser et al., 2004; Kuwaki et al., 2005; Tseng et al., 2005; Shimizu et al., 2008, Kim et al., 2013). Major features of thrombotic microangiopathy such as platelet activation, adherence, and clumping were inevitable consequences of immune-mediated transplant rejection (Rocha et al., 2003; Zhang et al., 2008). Thus, a disordered thromboregulation represents major immunological obstacles to long-term survival of xenoorgans. One of the reasons to causing blood coagulation in the tissue of xenografted organs was known to incompatibility of the blood coagulation and anti-coagulation regulatory system between TG pigs and primates (Cowan and d'Apice, 2009; Lin et al., 2009; Ekser et al., 2012).
Extracellular nucleotides (adenosine triphosphate; ATP and adenosine diphosphate; ADP) regulate thrombosis and inflammatory responses. ATP and ADP are converted to adenosine monophosphate (AMP) by CD39 (ectonucleoside triphosphate diphosphohydrolase 1; ENTPDase-1), which is catalyzed to adenosine by CD73 (ecto-5′-nucleotidase; e5NT) (Crikis et al., 2006). Decreasing of CD73 activity leads to reduce adenosine production and to decrease expression of cytoprotective factors and disordered blood coagulation control (Daval et al., 1991; Delikouras et al., 2003; Sitkovsky et al., 2004). In fact, pig endothelial cells in heart have significantly lower levels of CD73 compared with those of humans, resulting in decreased adenosine production in blood (Khalpey et al., 2005). For this reason, transplantation of GT KO pig organs expressing human CD39 and CD73 into the primates is expected to inhibit the inflammation reaction and blood coagulation (Cooper et al., 2012).
In our previous study, we constructed a human CD73 expression vector under control of porcine Icam2 promoter (pIcam2-hCD73) and established forty three donor cell lines expressing hCD73 (Oh et al., 2016). This study was performed to produce and breed transgenic pigs expressing hCD73.
Materials and Methods
Our study protocol and standard operating procedures for the treatments of the pigs used were reviewed and approved by the Institutional Animal Care and Use Committee of the National Institute of Animal Science, RDA (Approval number: NIAS2016-199, D-grade).
Our procedure for vector construction was previously described in detail (Oh et al., 2016). Briefly, the human CD73 cDNA clone was purchased from 21C Frontier Human Gene Bank (Korea, Clone ID, Hmu001092). The gene was synthesized by PCR using PrimeSTAR™ HS DNA polymerase (Takara Bio Inc, Shiga, Japan). That was amplified using upstream primer 5’-ATGCGGCCGCTTTCG CACCCAGTTCAC-3’ and downstream primer 5’-AATCT CTCGAGCTATTGGTATAAAACAAAG-3’, each of which has the restrict enzyme sites Not I and Xho I at the end of the sequence, respectively. The porcine Icam2 promoter was isolated by PCR from the template genomic DNA extracted from porcine ear tissue. The primers used for PCR were 5′-TATCGCGATCTAGAAACACACTGCAAG TCC-3′ and 5′-ATAAGCTTAGGAAAGAGCCGAGGAC CGCCT-3′ which contain Nru I and Hind III site, respectively. The pCMV-hCD73 vector was constructed by cloning the NotI and Xho I fragment of synthesized CD73 cDNA into pcDNA3.1 hygro vector. To generate another form of pIcam2-CD73 vector, the pCMV-hCD73 vector was digested into two segment using Nru I and Hind III, and then removed the CMV promoter. The Nru I and Hind III fragment of Icam2 promoter was inserted into the Nru I and Hind III site of hCD73 expression vector.
To introduce the exogenous DNA into the porcine endothelial cells originated from the main artery of 3-week-old male MGH (Massachusetts General Hospital) minipigs (generously donated by Dr. David H. Sachs, Transplantation Biology Research Center, Massachusetts General Hospital, Boston, Massachusetts 02129, USA), nucleofection was performed using primary mammalian endothelial cells, Amaxa Basic Nucleofector® Kit (Lonza). 48 hours after nucleofection, the cells were incubated in the medium contained 400 mg/mL of hygromycin. For the gene analysis of the clones selected by the hygromycin resistances, some of the cells were lysed for 2 hours at 55℃ with solution consistent of 50 mM KCl, 10mM Tris–HCl [pH 8.3], 2 mM MgCl2, 0.45%(v/v) NP-40, 0.5%(v/v) Tween 20, and 100 mg/mL of proteinase K. The insertion of pCMV-hCD73 vector was detected by PCR with upstream primer 5’-CTATTGACGTCAATGACGGTA-3’ and downstream primer 5′-CAAAACCCGAATGTCCCAGTGCAA-3′. To confirm the integration of pIcam2-CD73 vector into the endogenous genomic DNA, the gene was amplified by upstream primer 5′-ACTTAGTCATGGTGACTGCATGCC-3′ and the downstream primer which was same as previous one. The amplification conditions were as follows: initial denaturation at 94℃ for 5 min, 35 repetitions at 94℃ for 30s, annealing at 60℃ for 30s, extension at 72℃ for 1 min 30s, and then final extension step at 72℃ for 7 min. The amplified product size was 1 kb. After the confirmation, the transgenic donor cells were cultured in Dulbecco’s modified Eagle medium (WelGene Inc., Korea) containing 20% FBS, 1mM of non-essential amino acid (GIBCO), 1 mM of sodium pyruvate (WelGene, Korea), 55 μM of β-mercaptoethanol (GIBCO), and 1% antibiotics (100 U/mL penicillin and 100 μg/mL streptomycin; GIBCO) at 37℃ in an atmosphere of 5% CO2 in air and cryopreserved into LN2 until use.
In vitro maturation of porcine immature follicular oocytes was performed as follows with slight modifications (Hwang et al., 2015). Porcine ovaries were obtained from a local slaughterhouse (Nonghyup Moguchon, Gimje, South Korea) and transported to the laboratory at 30-35℃. Cumulus-oocyte complexes (COCs) were collected and washed in Tyrode's lactate-Hepes containing 0.1% (w/v) polyvinyl alcohol (PVA). Oocytes with several layers of cumulus cells were selected and washed three times in TCM-199 (GIBCO) supplemented with 0.1% PVA (w/v), 3.05 mM D-glucose, 0.91 mM sodium pyruvate, 0.57 mM cysteine, 0.5 µg/mL luteinizing hormone, 0.5 µg/mL follicle stimulating hormone, 10 ng/mL epidermal growth factor, 10% porcine follicular fluid (pFF), 75 µg/mL penicillin G, and 50 µg/mL streptomycin (maturation medium). For in vitro maturation, 50 COCs were transferred into 500 µL of maturation medium in a four-well dish (Nunc, Roskilde, Denmark). The oocytes were matured for 40 h at 38.5℃ under 5% CO2 in air. Somatic cell nuclear transfer was performed as follows (Kwon et al., 2017). Briefly, matured oocytes were enucleated by aspirating the first polar body and metaphase II chromosomes and a small amount of surrounding cytoplasm in manipulation medium supplemented with 5 µg/mL cytochalasin B. The freshly-thawed donor cells treated with Roscovitine were inserted into perivitelline space. The karyoplast-cytoplast complexes were placed into 0.2-mm diameter wire electrodes (1 mm apart) of a fusion chamber covered with 0.3 M mannitol solution containing 0.1 mM MgSO4, 1.0 mM CaCl2 and 0.5 mM Hepes. For reconstructions, two DC pulses (1-sec interval) of 1.5 kV/cm were applied for 30 µs using an Electro-Cell fusion (Fujihira Industry, Japan). Immediately after confirmation of fusion using a stereoscope, the reconstructed embryos were transferred into both oviducts of the surrogate (Landrace) on the same day or 1 day after the onset of estrus. Pregnancy was diagnosed on day 28 after ET and then was checked regularly every week by ultrasound examination. The TG cloned piglets were delivered by Caesarean section.
Genomic DNA (gDNA) was isolated from the tails of the cloned piglets. gDNA was used for transgene insertion by polymerase chain reaction (PCR) with specific primer sets using upstream primer 5′-TATCGCGATCTAGAAAC ACACTGCAAGTCC-3′ and downstream primer 5′-ATAA GCTTAGGAAAGAGCCGAGGACCGCCT-3′ which contain Nru I and Hind III site, respectively (Fig. 2A). The PCR cycle was as follows: initial denaturation at 95℃ for 5 min; 30 cycles of 94℃ for 30 s, the optimized annealing temperature for 30 s, and 72℃ for 30 s; followed by a final extension at 72℃ for 5 min.
Results
A summary of the pregnancy and delivery rate of hCD73-pig was described in Table 1. The mean number of embryos transferred was 127±18.9 of cloned embryos and 126±18.7 of parthenogenetic embryos, respectively. Among
No. of embryos transferred | No. of surrogates | No. of piglets | ||||
---|---|---|---|---|---|---|
NT | Parthenotes | Pregnancy | Delivery* | Live | Dead** | |
hCD73 | 127±18.9 | 126±18.7 | 8/18 | 3/18 | 3 | 7 |
18 surrogates, 8 were pregnant (44%) in early pregnancy period and 3 were maintained to full term (16%). The total number of delivered cloned piglets were 10 (2 alive, 7 mummy, and 1 died after birth) (Fig. 1A). The recipientbased individual cloning efficiency was range from 0.7% to 2.2% (Table 2). Produced transgenic pigs were confirmed by genomic PCR. All of the cloned pigs were confirmed as the hCD73 TG pig (Fig. 1B). And also the two hCD73 TG cloned pigs had normal reproductive ability. They mated with wild type (WT) MGH female sows and produced totally 16 piglets. Among them, 6 piglets were identified as hCD73 TG pigs (Table 3) (Fig. 2B).
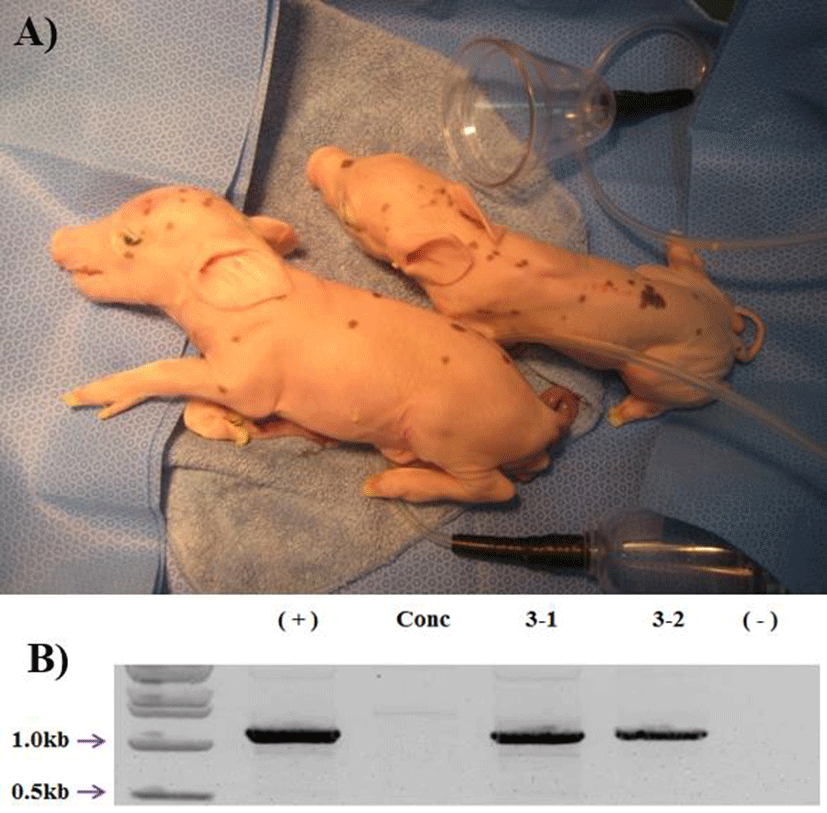
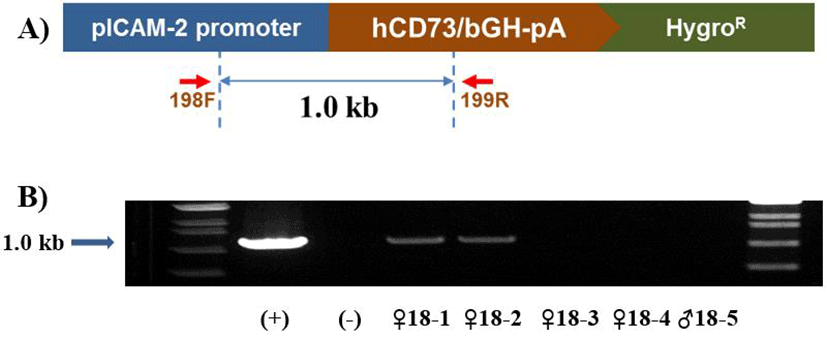
Discussion
Xenotransplantation results in hyperacute rejection mediated by the interaction with antibodies against α-Gal residues on cell surface glycoproteins of pig cells. The problem of hyperacute rejection has been overcome by homozygous GT KO pigs (Phelps et al., 2003; Kolber-Simonds et al., 2004; Nottle et al., 2007; Fujimura et al., 2008; Li et al., 2013). Although the transplantation of kidney or heart from a GT KO pig into an immunosuppressed primate could prolong the xenograft survival, the xenograft finally fails because of thrombotic microangiopathy that may be form of delayed immune-mediated rejection (Chen et al., 2005; Kuwaki et al., 2005; Lin et al., 2010).
Extracellular nucleotides and its derivatives directly or indirectly regulate many processes such as thrombosis or inflammation, vascular tone, and iron transport, via cell surface purinergic receptors (Arulkumaran et al., 2013; Zimmermann et al., 1996). However, the final effect of these signaling mechanisms is depended on the conversion of these nucleotides mediated by the cascade of ectoenzymes i.e. CD39 and CD73. These activities lead to the con-version of pro-inflammatory adenosine triphosphate (ATP) and adenosine diphosphate (ADP) to adenosine monopho-sphate and adenosine, which released from stressed or damaged cells into extracellular space. This change of ATP-dependent pro-inflammatory state into antiinflammatory environment is mediated by adenosine (Arulkumaran et al., 2013; Bauerle et al., 2011). It was reported that immune rejection in xenotransplantation experiments causes the loss of activity of porcine CD39 and CD73 resulting in a reduced capacity to convert pro-inflammatory and pro-aggregatory extracellular nucleotides into adenosine (Khalpey et al., 2005; Cowan 2007). Overexpression of both human CD39 and CD73 could enhance ATP and ADP breakdown and adenosine production in porcine xenotransplantation model (Cooper et al., 2012).
Wheeler et al. (2012) demonstrated that hCD39 transgene expression reduces myocardial injury in a porcine model of myocardial acute ischemia-reperfusion injury. In 2015, Iwase et al reported that transgenic GT KO pigs expressing complement regulatory protein (CD46) for inhibiting acute and vascular rejection, thrombomodulin and endothelial protein C receptor for repressing the blood clotting factors have been produced. Choi et al. (2016) produced the heterozygous GT KO transgenic miniature pigs expressing human CD39 for repressing the immune rejection responses that are common following by pig xenografts. Also, it has been reported that GT KO pigs heart overexpressing CD46 and thrombomodulin were transplanted into baboons and survived up to 945 days (Mohiuddin et al., 2016).
However, there has been no report on pigs that introduced hCD73, so the need for the development of transgenic pigs is being raised. Considering the function of sequentially degrading nucleic acids with CD39, it is assumed that the same effect will be obtained in transgenic pigs overexpressing CD73. However, it is not yet known whether adenosine produced by overexpressing CD39 or CD73 would effectively inhibit blood clotting when pig organs were transplanted into monkeys.
In this study, we successfully produced two healthy hCD73 transgenic cloned piglets and they have normal reproductive ability for next generation. In future, GT KO/ CD46/CD73 hybrid transgenic pig can be produced by crossing hCD73 positive and GT KO/CD46 TG pig and the TG pigs will be more effective model to use in research for controlling hyperacute and acute vascular rejection after xenotransplantation.