INTRODUCTION
Bone remodeling is a very dynamic process involving the coordinated phases of bone formation and resorption, which is based on a molecular crosstalk between osteoblasts involved in bone apposition and osteoclasts specialized in bone resorption (Ross, 2006; Filgueira, 2010). Receptor activator of nuclear factor kB ligand (RANKL) is expressed by osteoblasts and binds to its receptor RANK on the surface of osteoclasts and osteoclast precursors (Udagawa et al., 2000). These results lead to osteoclast formation, differentiation, activation and consequently bone resorption. To regulate the balance between bone formation and bone resorption, the RANKL-RANK interaction is inhibited by OPG. OPG produced by osteoblasts acts as a decoy receptor, binding to the RANKL, thus preventing its binding to RANK and subsequent osteoclast activation. As a result, bone resorption is inhibited (Reid & Holen, 2009). This triad of proteins, OPG/RANKL/RANK, has been shown in genetic and pharmacology studies to have a critical role in the regulation of osteoclasts and bone resorption (Kostenuik, 2005; Heymann, 2012).
Osteoprotegerin (OPG) is a secreted glycoprotein and a novel member of the tumor necrosis factor receptor (TNF-R) superfamily. The critical function of OPG in osteoclastogenesis has been initially revealed by the osteopetrotic phenotype of mice overexpressing it (Lacey et al., 1998; Yasuda et al., 1998). In contrast, OPG deficient mice exhibit osteoporotic phenotype which is totally reversed by administration of recombinant OPG (Min et al., 2000). These results point toward the potential utility of OPG in the treatment of many bone diseases associated with increased bone loss including post-menopausal osteoporosis, inflammatory arthritides, and metastatic bone tumors (Kostenuik, 2005; Anandarajah, 2009).
Transgenic animals are common and useful tools because they give an in vivo look at the ability and impact of foreign gene expression in a biological system (Maga & Murry, 1995). Production of pharmaceutically active human proteins in the mammary gland of transgenic animals has advanced to the stage of commercial application (Dyck et al., 2003). The mammary gland is the preferred production site mainly because of the quantities of protein that can be produced in this organ and established methods for extraction and purification of the respective protein (Rudolph, 1999). Several products derived from the mammary glands of transgenic goats and sheep have progressed to advanced clinical trials (Echelard et al., 2006; Kues & Niemann, 2011). Phase III trials for antithrombin III (ATryn from GTC-Biotherapeutics, USA) produced in the mammary gland of transgenic goats have been completed and the recombinant product has been approved as drug by European Medicines Agency in 2006 and in USA by FDA in 2009.
In this study, we designed a novel mammary gland-specific hOPG cDNA expression cassette by modifying the goat β-casein/hGH hybrid gene construct, which previously showed a potent and mammary gland-specific expression of hGH in transgenic mice (Lee, 2006). Then we examined expression profiles and biological activity of recombinant hOPG protein expressed in transgenic mice harboring the hOPG cDNA expression cassette.
MATERIALS AND METHODS
For an efficient and mammary gland-specific expression of hOPG from its cDNA sequences, a cDNA expression cassette was designed by modifying the goat β-casein/hGH hybrid gene construct, which previously directed a strong and mammary gland-specific expression of hGH in transgenic mice (Lee, 2006). The goat β-casein/hGH hybrid gene construct consists of 5’ promoter sequence (5.5 kb) of goat β-casein gene and entire structural sequence (2.1 kb) of hGH gene (Fig. 1A). In order to modify this hybrid gene for hOPG cDNA expression, internal pvuII fragment (984 bp) between exon II and exon V of hGH gene was excised out and ATG codons at exon I and II were replaced with SalI and HpaI cloning sites by PCR using a primer set (5'-AGCTGTCGACGCTACAGGTAAG-3' and 5'-GGCCAGCTGGTGTTAACGATGGGCGCGGAGGAT AGCG-3'). This modification generated a novel cDNA expression cassette (Fig. 1B) containing goat β-casein promoter sequences and exon I and II, intron I, poly (A), and 3’ flanking sequences of hGH gene with no ATG start codon. For hOPG expression, full-length hOPG cDNA (1,206 bp) was amplified by PCR using a primer set (5'-TCCCGGGGACCACAATGAAC-3' and 5'-GGTCGAC TTATAAGCAGCTTATTT-3') and inserted into HpaI site of the cassette by blunt ligation (Fig. 1C). Correct nucleotide sequence of this hOPG expression cassette was confirmed by sequencing before use for DNA microinjection.
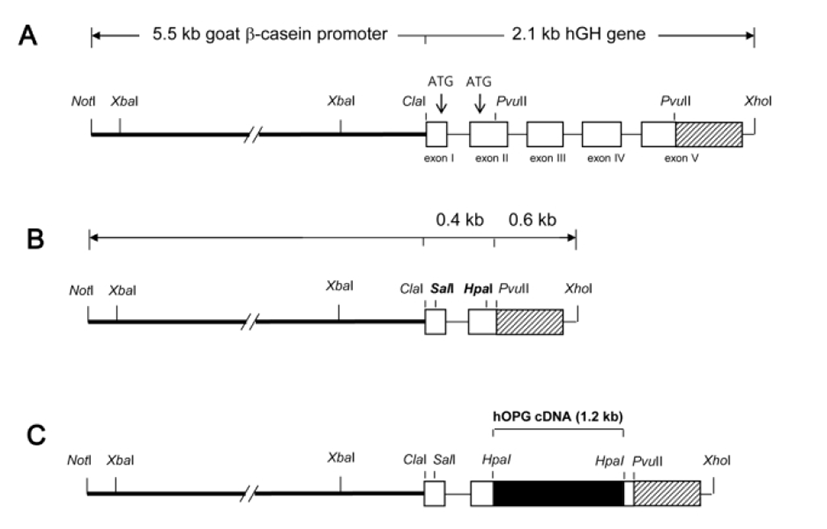
Transgenic mice were generated by a standard micro-injection method with a NotI/XhoI insert DNA fragment (Fig. 1C). Fertilized one-cell embryos from BCF1 (C57BL/6×CBA F1 hybrid, 8-week old) mice were micro-injected and transferred to the oviducts of pseudopregnant ICR females. Transgenic offspring were identified by PCR in genomic DNA samples from ear biopsies using a primer set (5'-AAGGAGCT GCAGTACGTCAA-3' and 5'-CAA TGTCTTCTGCTCCCACT-3') specifying hOPG cDNA sequences.
Milk samples were collected from founder transgenic females at 10 days of lactation as described previously (Oh & Lee, 2010) and their whey fractions were collected by a brief centrifugation. hOPG concentration in whey samples were determined by an hOPG-specific sandwich ELISA. In brief, a 96 well Immuno plate (Nunc, Denmark) was coated with mouse anti-hOPG antibody (R&D systems, USA) and then blocked with PBS containing 1% BSA, 5% sucrose, and 0.05% NaN3. After adding serial dilutions of whey samples or standard recombinant hOPG (R&D systems, USA), the plates were incubated with biotinylated goat anti-hOPG antibody. All binding reactions were conducted for 2 h at room temperature and unbound antibodies were thoroughly washed out following each reaction. To detect sandwiched hOPG proteins, streptavidine horse radish peroxidase and its substrate solution containing tetramethylbenzidine and H2O2 were sequentially added with 20 min interval. This reaction was terminated with 1M H2SO4 and optical density was measured at 450 nm in an ELISA reader (Titertek Multiscan model MKII, Labsystems, Finland). The concentration of hOPG in the milk was interpolated from the linear range (61.25 ‒ 3,200 pg/ml) of the standard curve.
Whey samples were also subjected to western blot analysis to determine the molecular weight of recombinant hOPG in milk. Whey samples were electrophoresed onto a 10% SDS-polyacrylamide gel and transferred to a nitrocellulose membrane (Schleicher & Schuell, Germany). The membrane was blocked with 3% BSA in PBS-T (PBS containing 0.1% Tween 20) and then incubated with 0.2 μg/ml biotinylated goat anti-human OPG IgG (R&D systems, USA) for 1 h at room temperature. After washing three times with PBS-T, the membrane was incubated with 1/200 diluted streptavidin-horse radish peroxidase conjugate for 30 min at a room temperature and washed again. The hOPG-specific binding was detected using ECL Plus (Amersham Pharmacia Biotech, USA) and visualized on X-ray film.
To determine tissue specificity of hOPG transgene expression, total RNA samples were isolated from various organs of 10 days-lactating mice using Trizol reagent (InVtrogen, USA). Total RNA (20 μg) was separated on 1.0% agarose-formaldehyde gels and then transferred to nylon membranes (Boehringer-Mannheim, Germany). Northern blot hybridizations were performed with 32P-labeled probes specific to hOPG cDNA sequences.
A cell-culture system allowing osteoclast development from hematopoietic precursor cells was used to analyze the biological activity of the milk hOPG (Lacey et al. 1998, 2000). Macrophage-stimulating factor (M-CSF) and osteoprotegerin-ligand (OPG-L, a soluble RANKL) have been known to be essential factors for both osteoclast precursors and osteoclast formation from bone marrow cells (Wan et al., 2001). Bone marrow cells from 8-weekold male BCF1 mice were cultured for 6 days in α-MEM containing 10% FBS, 50 ng/ml recombinant murine M-CSF (R&D systems, USA), and 50 ng/ml recombinant OPG-L (Chemicon, Germany) in 96-well plates (1×105 cells/well) to stimulate osteoclast formation. To test whether milk hOPG could inhibit the osteoclast formation induced by M-CSF and OPG-L, a series of diluted milk samples was added into the culture together with M-CSF and OPG-L. Culture medium was changed every three days with freshly prepared medium. Osteoclast formation was measured by a TRAP-solution assay. On 6 days of culture, cell extracts were prepared and the activity of tartrate-resistant acid phosphatase (TRAP), a typical marker enzyme for osteoclasts, was quantitated by measuring the conversion of p-nitrophenyl phosphate to p-nitrophenol in the presence of sodium tartrate using acid phosphatase activity assay kit (Sigma-Aldrich, USA) and expressed as optical density at 405 nm (Lacey et al., 1998). The experiments were carried out in triplicates.
RESULTS
Among thirty six offspring obtained from DNA-injected mouse fertilized eggs, twelve were identified as transgenic mice by PCR. Six transgenic females of them were bred to obtain lactating mothers. Milk samples were collected at 10 days of lactation. hOPG concentration and its molecular integrity in the milk samples were determined by ELISA and western blot analysis, respectively. Concentration of milk hOPG was 0.06 ‒ 2000 μg/ml in the six mice (Table 1). In particular, #5 and #6 transgenic mice highly expressed hOPG at the level of 1.6 mg/ml and 2.0 mg/ml, respectively. SDS-PAGE and western blot analysis revealed that the molecular weight of milk hOPG protein is approximately 55 kDa (Fig. 2), which is the same size as a naturally glycosylated hOPG monomer in cell cultures (Simonet et al., 1997), and relative signal intensity of samples #5 and #6 was comparable to their concentrations determined by ELISA (Table 1). These results demonstrate that the hOPG cDNA expression cassette efficiently produces recombinant hOPG with normal molecular weight in the milk of transgenic mice under the control of a modified goat β-casein/hGH hybrid gene.
Transgenic females | OPG concentration (µg/ml) |
---|---|
#1 | 2 |
#3 | 0.15 |
#4 | 0.2 |
#5 | 1,600 |
#6 | 2,000 |
#7 | 0.06 |
Non-transgenic control | Not detected* |
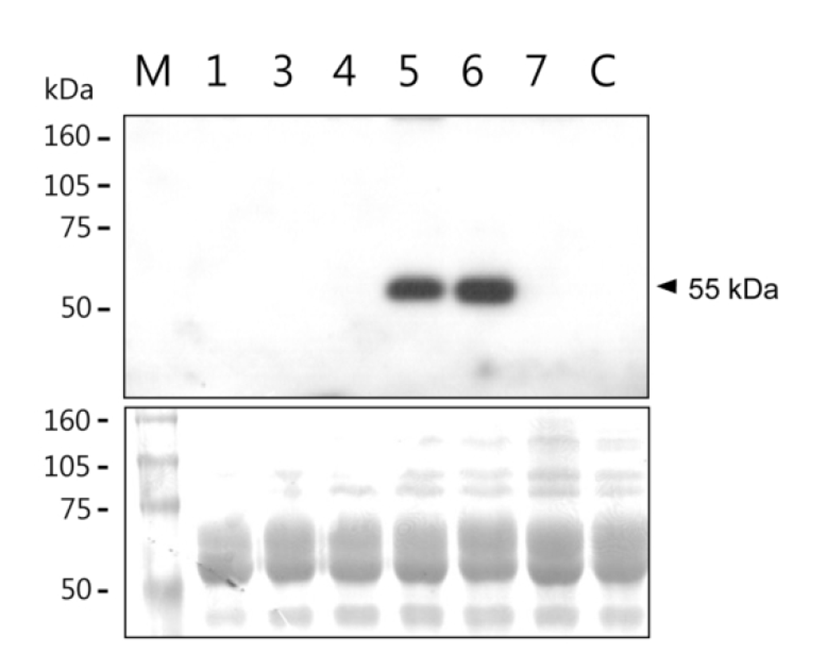
Tissue specificity of hOPG transgene expression was examined using total RNA samples isolated from various organs of highly expressing transgenic mice (#5 and #6) at 10 days of lactation (Fig. 3). Northern blot analysis revealed that hOPG transgene expression is highly specific to the mammary glands and its transcript is approximately 1.7 kb long. There were no signals in other organs of transgenic mice and all organs examined in control mouse. The signal intensity of sample #6 was stronger than that of #5. These results demonstrate that hOPG transgene expression directed by goat β-casein/hGH hybrid gene is highly specific to the mammary glands of transgenic mice.
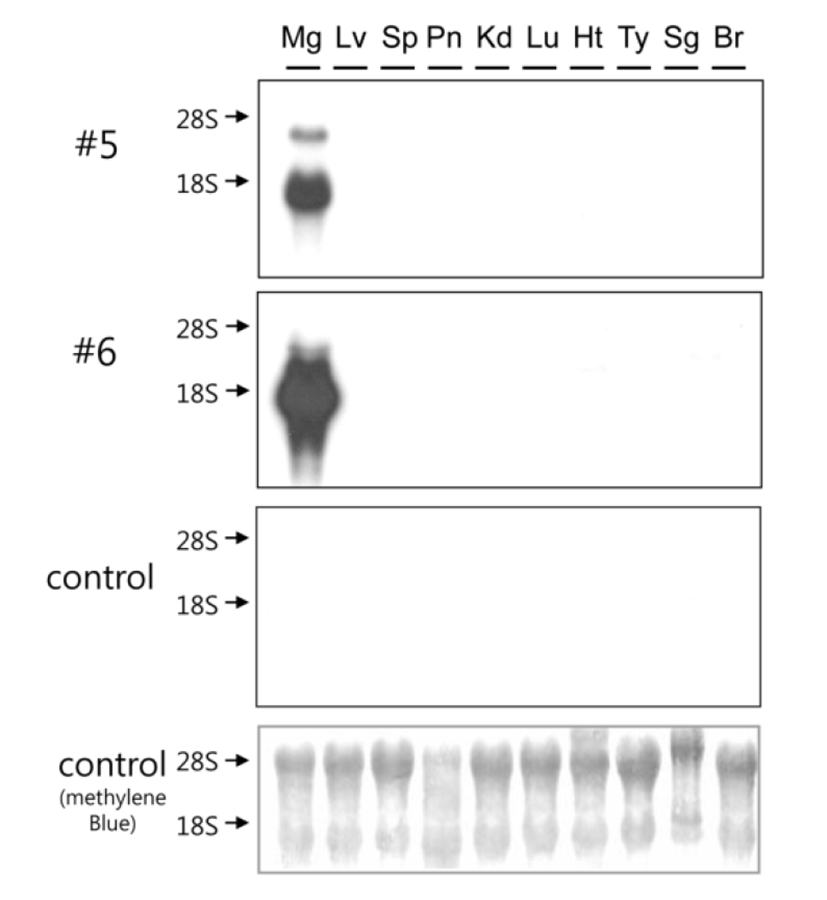
To examine the biological activity of hOPG in milk, a series of diluted milk together with M-CSF and OPG-L was added into bone marrow cell cultures for 6 days and then TRAP activity of the cell extract was measured. M-CSF/OPG-L treatment increased TRAP activity by two fold (Fig. 4A). However, co-treatment with transgenic milk samples (#5 and #6), not with control milk, inhibited this increase in a dose-dependent manner and 2 ng/ml hOPG in milk was sufficient for the complete inhibition (Fig. 4B). These results demonstrate that recombinant hOPG in the milk of transgenic mice has a normal biological activity which prevent OPG-L binding to RANK and subsequent osteoclast activation.
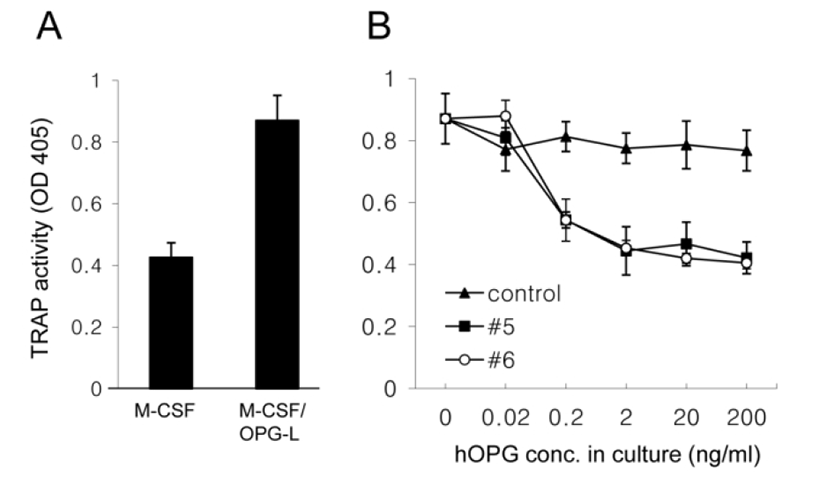
DISCUSSION
In this study, we designed a novel mammary gland-specific cDNA expression cassette and examined its expression in transgenic mice. hOPG cDNA was successfully expressed in the milk of all six transgenic mice analyzed and particularly two of them directed a high-level hOPG expression in a mammary gland-specific manner. Although full genomic sequences are generally better than intronless cDNA sequences for an efficient expression in transgenic animals (Rudolph, 1999), we used cDNA sequences for hOPG expression because its genomic sequence is too long (>29 kb) to manipulate easily. In fact, we modified the goat β-casein/hGH hybrid gene construct for cDNA expression because this hybrid gene previously directed high-level and mammary gland-specific expression of hGH in most transgenic mice examined (Lee, 2006; Oh & Lee, 2009, 2010). A part of hGH gene between exon II and exon V was excised out and two start codons in exon I were replaced with cloning site for cDNA insertion, resulting in hybrid minigene construct containing the first intron, poly(A) signal, and 3’ flanking sequences of hGH. Many minigene constructs have successfully expressed in transgenic mice (Persuy et al., 1995; Yan et al., 2006). The role of intron sequences for transgene expression was well studied in transgenic mice using GH gene and revealed that its first intron is essential for an efficient transgene expression (Palmiter et al., 1991). Introns could improve transgene expression by a number of different mechanisms (Palmiter et al., 1991). Some introns contain enhancers or cis-acting elements that influence transcriptional initiation or elongation and some contain sequences that facilitate opening of chromosomal domains, which could affect nucleosome composition and higher-order packing. Alternatively, mRNA splicing process may enhance mRNA stability in the nucleus. As we had expected, two transgenic mice strongly expressed hOPG from its cDNA sequences only in mammary glands of transgenic mice (Fig. 2 and 3). However, the level of hOPG expression in the other four mice was relatively very low when compared to that of highly expressing mice (Table 1). There could be two possible reasons for the variability of hOPG expression depending on the transgenic mice. One might be a position side effect from random integration of transgene. The other could be the loss of sequence elements included in the deleted part of hGH gene and/or loss of interaction between the deleted sequences and other regulatory elements essential for the consistent high-level expression of hGH gene. To test the second possibility, now we design another new cDNA expression cassette using entire hGH gene without deletion and will examine its expression profiles in cell cultures and transgenic mice.
Although hOPG expression was highly specific to the mammary glands of transgenic mice, we could not rule out its possible ectopic expression because very low level of hOPG was detected in blood of lactating hOPG transgenic mice (data not shown). However, we could not confirm this possibility in mRNA level because there were no northern signals for hOPG mRNA in any organs examined except mammary glands (Fig. 3). There are also other reports showing a presence of some milk proteins in blood of lactating normal animals (Aker et al., 1986; Grabowski et al., 1991). Therefore we exactly do not know whether the blood hOPG came from its ectopic expression or normal translocation from mammary glands. Whatever the reason is, blood presence of pharmaceutical proteins in transgenic animals must be carefully considered because of its possible detrimental effects on animal physiology. Recently, gene targeting and inducible expression methods, alternatives to direct DNA microinjection, are recommended to reduce possible variation in tissue specificity and expression efficiency in transgenic animals (Kues & Niemann, 2011).
For the clinical trials of recombinant proteins and its subsequent commercial production, their biochemical and biological properties are of great importance because natural and recombinant proteins often exist under different forms. This is particularly the case as far as glycosylation is concerned. Natural hOPG is a glycoprotein secreted as a monomer with 55 – 60 kDa or as a disulfide-linked homodimer and its deglycosylated hOPG monomer is about 40 kDa under reducing condition, which is predictable size from its cDNA sequences (Simonet et al., 1997; Tsuda et al., 1997). We also found 110-kDa dimmers as well as 55-kDa monomer in transgenic milk samples under non-reducing condition (data not shown) and they inhibited TRAP activity induced by OPG-L in a similar dose range with purified recombinant hOPG from cell cultures (Tsuda et al., 1997). Therefore it could be demonstrated that hOPG in our transgenic milk is properly glycosylated and its normal biological activity revealed by TRAP activity assay might be attributed to its normal glycosylation pattern (Liu et al., 2003).
The balance between bone resorption and bone apposition depends on the ratio OPG/RANKL. From the strong evidences for anti-resorptive activity of OPG, many preclinical trials have long been done to evaluate the potential utility of OPG in the treatment for many bone diseases associated with increased bone loss (Anandarajah, 2009). However, recently the role of OPG in bone cancer remains contro-versial, as this protein also binds to TRAIL (TNF related apoptosis inducing ligand), another member of the TNF superfamily, resulting in the inhibition of tumor cells apoptosis (Lamoureux et al., 2010). In these circumstances, OPG could become a survival factor for tumor cells. Therefore the application of OPG for the treatment of osteolysis should be carefully considered depending on the pathophysiological conditions especially oncological diseases and further understanding of OPG biology will be necessary for its successful clinical applications.